Repurposed gas reservoirs, pipelines increase hydrogen applications
Depleted natural gas reservoirs and existing gas pipelines are options for hydrogen storage and transport. Hydrogen’s unique properties, however, require specific analyses of equipment and reservoirs.
Efficient hydrogen storage and production depends on the timing of hydrogen injection into a depleted reservoir. A study to covert a partially depleted natural gas reservoir in Ordos basin, China, showed that injecting hydrogen in a reservoir earlier during depletion results in lower hydrogen purity in the recovered gas but higher hydrogen recovery due to additional available pressure. Cyclic hydrogen injection-withdrawal results in increasing hydrogen production after each cycle with minimal molecular diffusion into the reservoir under high injection rates.
A review of Snam SPA’s natural gas pipeline network examined compliance of the existing system for hydrogen service and identified gaps requiring further analysis. The study concluded that pipelines constructed after the year 2000 require limited technical evaluation, and about 1,148 km of SNAM’s existing pipelines are suitable to transport 100% pure hydrogen.
Hydrogen storage
Hydrogen has high mass-energy density (120 megajoule/kg) but low density (0.08375 kg/cu m). The low density requires large storage volumes such as found in salt caverns and depleted reservoirs. Hydrogen also has low solubility in water and low dynamic viscosity, leading to potential leakage from underground containment.
Salt caverns require large cavities, and creating those from freshwater injection and circulation can take up to 5 years. Existing depleted reservoirs, by contrast, provide ready storage and existing topside infrastructure which can be repurposed for hydrogen service. Hydrogen has low adsorption on the carbonate rocks and sandstones typically found in hydrocarbon reservoirs. These reservoirs, however, must have suitable cap layers and a complete trap structure to contain hydrogen and prevent upward migration.
Hydrogen can dissolve into oil; therefore, depleted gas reservoirs are superior to oil reservoirs for this service. The remaining gas in depleted gas reservoirs also provides a gas cushion to act as a barrier between hydrogen and the natural fluids present in the reservoir. The gas cushion also pressurizes the reservoir to maintain the desired production rate. Hydrogen, nitrogen, and carbon dioxide serve as cushion gases in the absence of reservoir gas. Other cushion gases must be analyzed for compatibility with existing reservoir fluids to prevent unwanted reactions. If cushion gases co-produce with hydrogen, topside separation will be required.
Seasonal hydrogen storage-production simulations confirmed that hydrogen storage in depleted gas reservoirs was feasible and had less than 0.1% hydrogen loss through dissolution and diffusion. Microbial activity and molecular diffusion, which is hydrogen lost to the reservoir due to diffusion into water, resulted in only minor reductions in reservoir rock porosity. A North Sea hydrogen storage numerical study estimated 87% final hydrogen recovery with maximum recovery achieved by pre-injecting formation gas as cushion gas. Mixed formation gas and hydrogen lead to lower hydrogen purity. By contrast, depleted condensate reservoir studies showed that nitrogen cushion gas yielded the highest hydrogen recovery and purity. Optimal hydrogen storage came from condensate reservoirs with 60% depletion.
Ordos basin repurposing
A numerical simulation analyzed the hydrogen storage capacity of a partially depleted natural gas reservoir in “T” field in Ordos basin, China. The gas reservoir covers a 1.8-km × 1.9-km area with 2,000-m reservoir depth and 430-m reservoir thickness. The reservoir was developed using two vertical wells (Fig. 1). Rock compressibility is 1 × 10-5 kPa-1. Average reservoir pressure was 7 megapascals (MPa) at the beginning of hydrogen storage operations, representing 65% original gas recovery.
The reservoir was modeled with 35 × 36 × 46 grid cells in x, y and z directions, respectively. Table 1 summarizes T-field properties for the simulation. Hydrogen serves as the primary component of the injected gas, with hydrogen, nitrogen, carbon dioxide, and methane as cushion gases before hydrogen injection.
After simulating 16 years of primary gas depletion at 0.4 × 106 cu m/d to 7 MPa, hydrogen storage simulations ran for 7 years, with a final 7-year production period for a total simulation of 30 years. Ten hydrogen storage-extraction cycles were run with each cycle including 6 months of hydrogen injection and 3 months of gas production. Hydrogen injection was 1 × 106 cu m/day and gas extraction was twice the injection rate at 2×106 cu m/d. Sensitivity analysis optimized injection timing, injection-production cycles, injection rates, molecular diffusion, and various cushion gases on hydrogen storage and extraction.
For the base case, hydrogen recovery reached 74.36% after 10 injection-production cycles and produced 92.1% ultimate hydrogen recovery after ending injection. Adding a gas cushion improved recovery, with nitrogen and methane providing the best response. Using hydrogen for cushion gas resulted in the highest reservoir pressure at 9.95 MPa. Substituting nitrogen cushion gas resulted in the next highest reservoir pressure at 9.75 MPa, followed by methane at 9.49 MPa and carbon dioxide at 9.01 MPa. Ultimate hydrogen recovery inversely followed these pressure trends.
The effects of reservoir depletion on recovery were simulated for reservoirs depleted 1 MPa to 13 MPa below the 20 MPa initial reservoir pressure (19.7-MPa reservoir pressure, respectively). Ultimate recovery for these cases increased with initial reservoir pressure, reflecting the additional energy provided to the system with the higher pressure (Table 3). Hydrogen purity, however, suffers as the lower levels of depletion leave more methane in the reservoir to mix with the hydrogen gas. Methane must be removed at surface and adds cost and complexity to the process. Hydrogen storage optimization, therefore, must balance the desire to increase recovery efficiency with purity.
With respect to molecular diffusion on hydrogen recovery, the simulations indicate that diffusion is inversely injection-rate dependent. At high injection rates, diffusion has a small impact on hydrogen storage. Simulations run with a lower injection rate than in the base case showed that diffusion reduced hydrogen recovery by up to 3.2%. Fig. 3 shows hydrogen diffusing away from the injection well and further into the reservoir under low-rate injection. Diffusion also reduces the purity of produced hydrogen because it mixes with existing reservoir gas, and hydrogen production and recovery subsequently decrease.
Pipeline repurposing
Extensive cracking from preexisting flaws or cracks represents the main threat to existing pipelines from hydrogen service during hydrogen-environment assisted cracking (HEAC) (Fig. 4). Hydrogen dissociates and absorbs at crack tips, embrittling the crack front. Fracture occurs at sustained loads below the yield strength of the material and fracture development optimizes near room temperature for typical pipeline metallurgy. Carbon and low-alloy steels exhibit fatigue crack growth even at relatively low hydrogen gas pressure. Crack growth increases under stress. Under high pressure, hydrogen will reduce tensile strength and ductility even without the presence of a defect.
Depending on the pipe material and hydrogen partial pressure, steel decreases in ductility by 20-80% and in fracture toughness by 35-70%. Cyclic loading from storage and withdrawal will exacerbate fatigue. Crack growth will not occur, however, if the environment is kept below a defined critical-stress intensity.
There are no current European technical standards which directly address conversion of existing natural gas pipelines to hydrogen service. Table 4 lists other international standards and technical documents for hydrogen service requirements which can serve as guidelines for repurposing.
The ASME B31.12 code addresses design and operation of hydrogen pipelines but does not provide a practical path to hydrogen service repurposing. It penalizes grades above API 5L X52/L360 by allowing, with little technical evidence, only half the operating pressure in these pipes for hydrogen than for natural gas under certain circumstances. Almost half the natural gas pipelines in Europe and America use these grades.
Restrictions can be worked around, but only if destructive material sampling occurs every mile along the proposed converted pipeline. In this event, natural gas design factors can be used for hydrogen service, but this test requirement is impractical. For this reason, the Rosen Group proposed a pipeline repurposing roadmap to practically guide pipeline repurposing to long-term hydrogen service.
The roadmap consists of five stages:
- Phase 1. Definition of project boundaries.
- Phase 2. Development of conversion strategy and feasibility assessment.
- Phase 3. Establishment of risk profile.
- Phase 4. Conversion suitability assessment.
- Phase 5. Conversion-integrity management planning.
Phase 1 identifies the pipeline from start to finish and includes ancillary equipment such as pig traps and valves. Operational conditions and applicable codes and regulations are also identified.
Phase 2 searches for technical gaps and major barriers to conversion. An integrity overview identifies fitness for service of the existing pipeline and historical threats such as corrosion, sulfide stress cracking, damage, hard spots, etc. Maximum allowable operating pressure (MAOP) and expected pressure cycling during hydrogen service are determined. A review of regulations and codes, engineering analysis, critical gaps, and technical feasibility of repurposing leads to a technical and economic risk assessment.
Phase 3 addresses critical gaps and assumptions from Phase 2 to produce a pipeline conversion-risk profile assessment to compare against operational and financial expectations. This is the phase in which the 1-mile destructive testing requirement in ASME B31.12 is addressed. Pipeline grade-determination tools identify pipe properties such as yield strength, grade, and records (Fig. 5). Destructive testing and additional actions are targeted at specific locations of concern rather than every mile.
Phase 4 confirms MAOP, compliance against applicable codes and regulations, risks, and project roadblocks. Pipeline conversion and operational planning begin after all objectives are met. Phase 5 develops an integrity management program which includes inspection, monitoring, and maintenance.
SNAM pipeline
RINA Co. performed a hydrogen service material assessment of the existing pipeline network operated by Snam, Italy’s main gas transmission system operator, with more than 30,000 km of pipelines in service. The study defined technical acceptance criteria and developed a method to evaluate pipeline repurposing.
Fig. 6 illustrates a flowchart for assessing conversion of natural gas pipelines to hydrogen service. It contains three elements which echo the Rosen Group’s phased approach:
- Define technical requirements for hydrogen service.
- Determine technical and engineering documentation required to satisfy hydrogen service technical requirements.
- Define material testing requirements, if necessary.
Line pipe fabrication, material grade, chemistry, hardness, and toughness requirements are defined for all operating conditions up to the MAOP. Additional chemistry, tensile strength, and toughness requirements depend on whether the material operates at a hoop stress higher than 40% of the specified minimum yield strength (SMYS). There are no requirements for hydrogen environment testing, but without such testing the MAOP in hydrogen service may be lower than in natural gas service. Testing can raise the MAOP limit once test-acceptance criteria are defined and met.
RINA reviewed Snam’s natural gas pipeline network to determine compliance of the existing system with hydrogen service and identify gaps that may require testing or other study. Early Snam specifications are lacking to support hydrogen service, but the latest specifications are within compliance with most hydrogen-specific technical requirements.
Pipelines constructed after the year 2000 require only limited evaluation of technical aspects such as hardness of welds, as well as collection of additional documents. A review of pipeline properties up to first-quarter 2023 suggests that about 1,148 km of existing pipelines and welds operated by Snam can transport 100% pure hydrogen (Table 5).
Costs, impediments
DNV estimates that $180 billion will need to be spent on hydrogen pipelines between 2022 and 2050 to meet global carbon reduction goals, and this includes more than 50% of hydrogen pipelines coming from the repurposing of natural gas lines. The assurance and risk management company says that this number could be as high as 80% in some regions, driven by costs expected to be just 10-35% that of building a new pipeline.
Aside from the pipelines themselves, hydrogen’s extraction from storage also requires attention. Its lower density will require development of more rapid means of withdrawal to maintain appropriate pipeline operating pressures.
One method of addressing this would be to have a more broadly distributed storage network than has been required for natural gas. This is also important in terms of maintaining sufficient linepack in instances during which hydrogen would be mixed with natural gas for transport.
DNV describes pipeline transportation of hydrogen as “robust and inexpensive for distances up to 2,000 km” (1,240 miles).
Ammonia can be transported more cheaply, but removing the hydrogen from it requires significant energy, at least partially defeating the purpose. It is also toxic if accidentally released.
Bibliography
DNV, “Hydrogen Forecast to 2050,” June 14, 2022.
Dong, Z., Wei, X., Zou, L., Yang, Z., Hou, B., Hou, T., Li, W., Lin, K., Yi, H., and Liu, Z., “Numerical Simulation Study of Hydrogen Storage in the T Gasfield in Ordos Basin,” URTeC-396912, Latin America Unconventional Reserouces Technology Conference, Buenos Aires, Argentina Dec. 4-6, 2023.
Martinez, O.R., Naib, S., Sandana, D., and Gallon, N., “A Practical Guide to Repurposing Existing Pipeline for Transporting Hydrogen,” OMC-2023-445, 16th OMC Med Energy Conference and Exhibition, Ravenna, Italy, May 23-25, 2023.
Tarantini, A., Mannucci, G., and Bacchi, L., “Repurposing of Existing Natural Gas Pipelines for Hydrogen Service – a Methodology for Third-Party Suitability Assessment for a Material Perspective,” OMC-2023-456, 16th OMC Med Energy Conference and Exhibition, Ravenna, Italy, May 23-25, 2023.
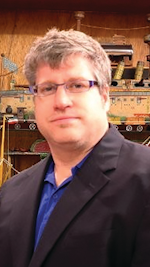
Alex Procyk | Upstream Editor
Alex Procyk is Upstream Editor at Oil & Gas Journal. He has also served as a principal technical professional at Halliburton and as a completion engineer at ConocoPhillips. He holds a BS in chemistry (1987) from Kent State University and a PhD in chemistry (1992) from Carnegie Mellon University. He is a member of the Society of Petroleum Engineers (SPE).